The industry must join a growing chorus in calling for new technology.
Steven Letendre is associate professor at Green Mountain College. Contact him at letendres@greenmtn.edu. Paul Denholm is an energy analyst at National Renewable Energy Laboratory. Contact him at Paul_Denholm@nrel.gov. Peter Lilienthal is senior economist at National Renewable Energy Laboratory. Contact him at peter_lilienthal@nrel.gov.
The prospect of millions of vehicles plugging into the nation’s electric grid in the coming decades never has been better. In 2005, hybrid electric vehicles (HEV) reached 1.2 percent of new cars sold in the United States, more than doubling the number sold in the prior year. Vehicle manufacturers, betting on this trend accelerating in the coming years, are rushing to bring HEVs to their dealers’ showrooms.
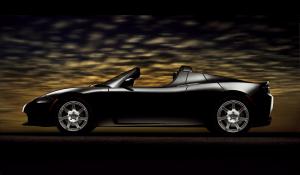
The evolution of HEVs to allow charging from the electric grid—so called plug-in hybrids (PHEV)—is assumed by many to be desirable, even inevitable. Indeed, a growing movement to bring PHEVs to market has emerged, bolstered by the undeniable economic and national-security benefits that result from displacing gasoline with electricity.
One highly visible grassroots campaign called Plug-In Partners seeks to demonstrate to the major automobile manufacturers that a national market exists for flexible-fuel PHEVs; dozens of businesses, utilities, municipal governments, and environmental groups have joined the Plug-In Partners campaign.