Can nuclear heat allow for low-cost commercial reclamation?
Michael F. Donnelly, Ph.D. is vice president, strategic consulting at Ventyx. Email him at Mike.Donnelly@ventyx.com.
Deposits of unconventional fuels—both crude oil and natural gas—occur in geological environments with very low energy. In oil shales, such fuels initially were deposited as hydrocarbon material called bitumens in host sedimentary rocks. In oil sands, the bitumens migrated from their original depositional environment into more porous deposits.
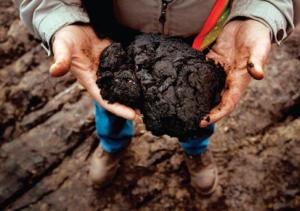
Such deposits, whether asphaltic sandstones in western Canada (tar sands) or pyrobituminous shales in the western United States (oil shales), have very low fluid transmissibility reflecting in part the compaction of fine-grained detritus and their absorption of hydrocarbons. The exploitation of these low-energy deposits/reservoirs will require significant external energy to replace that lost or never provided by Mother Nature’s handiwork. Such input of energy must reflect the geological nature of the deposit, which largely will determine the nature of extraction (in-situ or mining) and of processing (upgrading and blending). To achieve a marketable product at a price that justifies significant long-term capital investments—both initial and sustaining—is the challenge facing the oil industry today. Bitumen-derived heavy oil is not strictly a commodity like lighter crudes. It sells at a discount (typically +/- $20/bbl) because it is unmarketable until dilutent pipelines, refineries, and product pipelines are built. The products are diluted bitumen blends and synthetic crude oil (SCO).